XUV Lasers & Applications
XUV lasers (typically from 1 to 50 nm wavelength) with plasma amplifiers are compact coherent sources that complement high-order harmonics and XUV free-electron lasers. They produce ultra-short pulses in beams of exceptional quality.
Principle: An ultrashort pump laser pulse is focused on a gaseous target, creating a plasma composed of multicharged ions and free electrons. Ionization is caused by the electric field of the laser which tears the electrons away from the atoms of the neutral gas while accelerating them. The electrons can then transfer energy to the ions through collisions. Under particular conditions and for certain specific ions (isoelectronic series of neon, nickel, or palladium), a population inversion can be obtained for a brief time. This results in a strong amplification of spontaneous emission (ASE). Since spontaneous emission is a stochastic phenomenon, the beam generated is very noisy, incoherent and divergent. The injection of a resonant high-order harmonic pulse into the amplifying medium, on the other hand, leads to a very strong improvement in the spatial characteristics of the beam.
In 2001-2002, we demonstrated the first saturated amplification of an ionized XUV laser by optical field ( S. Sebban et al., Phys. Rev. Lett. 86, 3004-3007 (2001) , Phys. Rev. Lett. 89, 253901 (2002) )
In 2004, we realized the first laser chain in the XUV domain by injecting the amplifier with a high-order harmonic pulse, which notably allowed the generation of high-quality beams with linear polarization (P. Zeitoun et al., Nature 431, 426-429 (2004) )
In 2009-2010, we demonstrated that the spatial filtering of the high-order harmonic injected into the amplifier allows to generate XUV laser beams limited by diffraction, while maintaining a very strong temporal coherence ( J.-P. Goddet et al., Opt. Lett.34, 2438-2440 (2009) , O. Guilbaud et al., Opt. Lett. 35, 1326-1328 (2010) )
In 2015,we performed the first amplification of a harmonic beam of circular polarization, and demonstrated experimentally that a strong increase in the density of the plasma amplifier led to the generation of sub-picosecond XUV ray pulses (A. Depresseux et al.,Phys.Rev Lett 115, 083901 (2015),Nature Photonics 9, 817-821 (2015))
In 2018, we successfully implemented a waveguide by focusing with an axicon lens a sequence of “igniter” (130 mJ, 30 fs) and “heating” (690 mJ, 600 ps) pulses delayed by 600 ps. When injecting a 5 × 10 18 W.cm-2 laser pulse into the waveguide, the electron density increases to more than 1020 cm-3 . The transmitted beam is multimode and contains about 50% of the initial energy after 5 mm of propagation and decreases to 20% after 20 mm when krypton is used. In parallel with the experimental measurements, intensive numerical calculations using hydrodynamic and particle codes in the cell have been performed to understand and predict the creation and subsequent evolution of the waveguide ( E. Oliva et al., Phys Rev.E 97, 023203 ( 2018), E. Oliva et al., Phys. Rev. A 92, 023848 (2015)
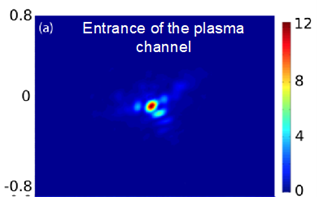
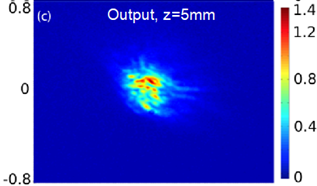
Fig. 1: Section of the intensity distribution of the infrared pump laser at the plasma inlet (left) and the outlet (right). The redder the image, the higher the intensity. The beam structure is well preserved thanks to the waveguide.
In 2020, we showed that stimulated emission can be triggered simultaneously on two transitions with similar gain dynamics. The amplifier was injected by a pulse containing two resonant high-order harmonics, thus producing a short-lived, coherent two-color laser beam (at 32.8nm and 62.7nm). F. Tissandier et al., Phys. Rev. Lett. 124 et 133902 (2020)
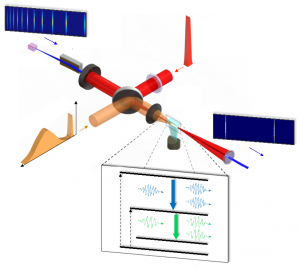
In 2020, in collaboration with Dr. Zürch’s team at the Institute of Optics and Quantum Electronics at the University of Jena, using a ptychographic approach, we were able to reconstruct the complex wavefront of the injected EUV laser wave. The EUV beam was focused on a sample consisting of a regular hole pattern. The coherently diffracted light is recorded in a transmission geometry and the sample is scanned using a ptychographic method. This allows the complex field to be retrieved in the sample plane, which has been backpropagated to the amplifier’s output plane. The backpropagated field has a parabolic phase profile and a two-peak amplitude profile that are related to the non-homogeneous gain distribution in the amplifier. This shows excellent agreement with 3D Maxwell-Bloch calculations.
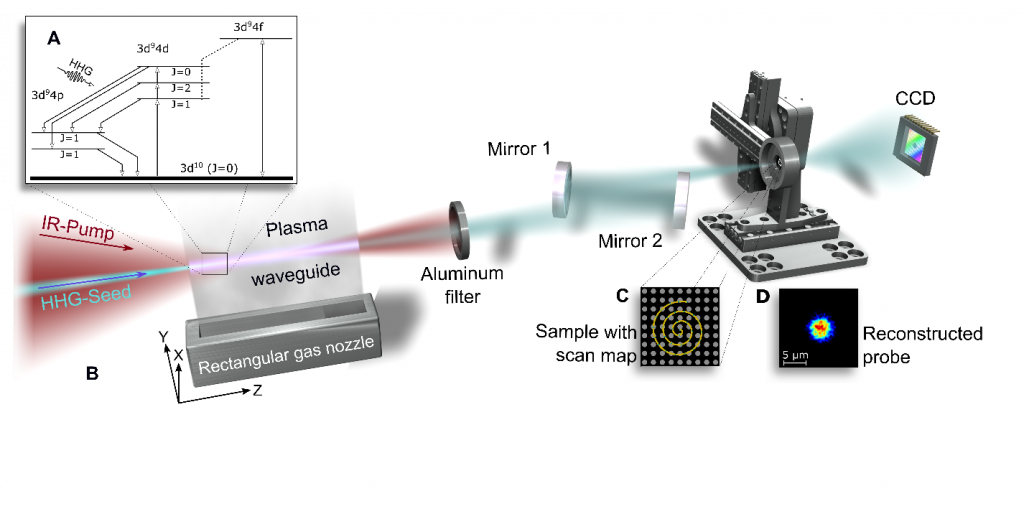
In 2021, in collaboration with the University of Berkeley (USA) and the University of Jena (Germany), we carried out the first frequency doubling in the XUV domain. By making the best use of the XUV laser source developed in the laboratory, we were able to achieve the high light intensities required for the occurrence of non-linear phenomena. The XUV pulse at 32.8nm was focused on the surface of a thin sheet of titanium, and observation of the transmitted radiation using a high-sensitivity spectrometer revealed the generation of the second harmonic of the XUV laser, at a wavelength of 16.4nm. Additional measurements and numerical calculations have shown that the non-linear conversion takes place on the surface of the titanium sheet, and that it has been significantly enhanced by the resonant absorption of the XUV laser pulse. The surface and element specificity of the XUV second harmonic generation process makes it a well-suited technique for studying the intrinsic properties of complex systems with a wide range of applications, such as all-solid-state batteries, ferroelectric materials, semiconductor heterojunctions, and other low-dimensional quantum heterostructures, to name a few. The results also point to the possibility of ultrafast nonlinear XUV spectroscopy using larger-scale sources. ( T. Helk et al., Sci. Adv. 7(21) abe2265(2021) )
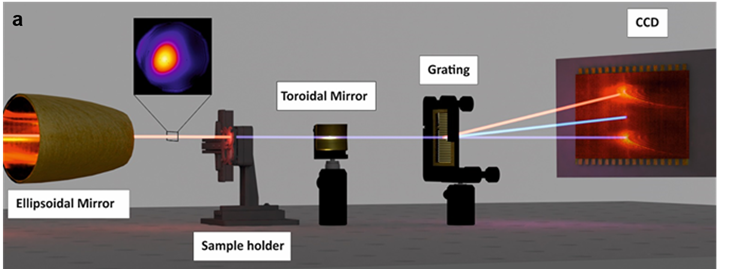
In 2022, we developed and used a measurement technique to extract the time profile of the XUV pulse in a single shot with a resolution of about 200fs. This technique is based on the sudden change in reflectivity of an XUV multilayer mirror following its irradiation by an fs laser beam. The single-shot operation is made possible by the in-line focusing of the laser beam allowing a gradual deposition of its energy along the line. We were able to measure that the duration of the XUV laser pulse is around 500fs. ( A. Kabacinski et al., Phys. Rev. Research 4, L032009 (2022) )
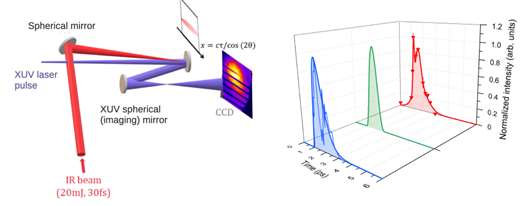
In 2023, we were able to control the group speed of the high-intensity laser pulse (I>1018 W/cm²) in the plasma thanks to the spatio-temporal couplings of the pump laser (flying focus effect), allowing in particular to further reduce the duration of the XUV laser pulse generated.
Negative plasma dispersion is indeed highly limiting in many laser-plasma interaction experiments performed at high density. In the case of XUV laser generation, it leads to a desynchronization between the infrared pump beam (vg < c), and the XUV beam (vg ≈ c), which considerably limits the effective amplification length. The measurements we have made have shown that maintaining the group speed of the pump pulse at a speed close to c optimizes the energy extraction in the XUV laser amplifier, but also reduces the duration of the XUV pulse generated in this amplifier. Using the technique presented above, we measured XUV laser pulses with an RMS duration of 350fs.
Dispersion compensation by space-time couplings offers great potential for optimizing the XUV laser source and opens up prospects for many applications requiring ultrashort pulses or high target intensities at short wavelengths. [A. Kabacinski et al. Nature Photonics 17, 354-359 (2023) ]